Leveraging genomics, transcriptomics and epigenomics to understand chemoimmunotherapy resistance in chronic lymphocytic leukemia
Abstract
Patients with chronic lymphocytic leukemia (CLL) have differing clinical outcomes. Recent advances integrating multi-omic data have uncovered molecular subtypes in CLL with different prognostic implications and may allow better prediction of therapy response. While finite-duration chemoimmunotherapy (CIT) has enabled deep responses and prolonged duration of responses in the past, the advent of novel targeted therapy for the treatment of CLL has dramatically changed the therapeutic landscape. In this review, we discuss the latest genomic, transcriptomic, and epigenetic alterations regarded as major drivers of resistance to CIT in CLL. Further advances in genomic medicine will allow for better prediction of response to therapy and provide the basis for rational selection of therapy for long-term remissions with minimal toxicity.
Keywords
INTRODUCTION
Recent large-scale whole-genome sequencing and multi-omics studies have revealed marked heterogeneity in chronic lymphocytic leukemia (CLL) and identified novel genetic and epigenetic alterations causing resistance to chemoimmunotherapy (CIT) in CLL, leading to a better understanding of the pathogenesis of CLL. While historical CIT combinations have allowed patients with CLL to achieve good responses formerly, targeted therapies (e.g., bruton tyrosine kinase and B-cell lymphoma-2 inhibitors) have impressive activity in high-risk genomic subgroups and have largely replaced the role of CIT for all patients with CLL in certain countries. Despite this, there may be a select group of patients aged less than 65 years old, with immunoglobulin heavy-chain variable region (IGHV)-mutated disease and without high-risk cytogenetic abnormalities including del(11q) and del(17p), in whom fludarabine, cyclophosphamide, and rituximab (FCR) can offer durable remissions, especially if financial toxicity is a concern[1].
While the role of CIT continues to diminish in frontline CLL, long-term follow-up of patients with mutated IGHV and without tumor protein p53 (TP53) aberrancy treated with FCR in a phase II clinical trial showed sustained progression-free survival (PFS) of 54% at 12 years, with no relapses observed beyond 10.4 years follow-up[2], suggesting FCR could cure a significant portion of patients with good risk disease. The CLL8 trial, which evaluated fludarabine/cyclophosphamide (FC) against FCR, also showed, at a median 5.9 years of follow-up, that some patients without del(17p) remained in long-term remission[3]. Lastly, the GAIA-CLL13 trial randomized young and fit patients with CLL without TP53 aberrations to receive FCR/bendamustine-rituximab, time-limited venetoclax-obinutuzumab, or ibrutinib with venetoclax-obinutuzumab, and found no PFS benefit among patients with mutated IGHV[4]. When selecting FCR as the treatment for frontline CLL, it is critical to carefully counsel the patient about potential toxicities, including cytopenias with concomitant infectious complications, and a small but increased risk (6.3%) of secondary acute myeloid leukemia/myelodysplastic syndrome[3]. In this review, we will detail the genomic, transcriptomic, and epigenetic alterations that portend poor outcomes in patients with CLL treated with CIT. These emerging mechanisms of resistance to CIT can guide clinical care and assist in the selection of the most appropriate therapy for patients with CLL.
GENOMIC ALTERATIONS AND CHEMOIMMUNOTHERAPY RESISTANCE
Common genomic alterations in CLL that are associated with chemotherapy resistance are detailed below and summarized in Table 1.
Recurrently mutated genes in CLL and mechanisms of chemoresistance
Biomarker | Mechanisms of resistance | Prevalence before treatment and at progression | Implications | Ref. |
Del(17p)/TP53 mutations | Genomic instability, survival advantage, and reduced DNA damage response | 5% to ~30% | Poor response to CIT | [5-8] |
Unmutated IGHV gene | Increased B-cell receptor signaling capacity | ~40% to 70%-80% | Poor response to CIT | [3,46] |
BIRC3 mutations | Upregulation of non-canonical NF-κB signaling pathway | 2%-6% to 8% | Poor response to CIT | [19-21] |
NOTCH1 mutations | Transcriptional activation of cell survival and proliferation and reduced expression of CD20 | 8%-10% to 30% | Poor response to CIT and anti-CD20 mAbs | [24-26] |
ATM mutations | Activates cell cycle checkpoints to induce apoptosis of cells with excess DNA damage | 20% to 30% | Poor response to CIT | [14] |
POT1 mutations | Increased chromosomal aberrations and genomic instability | 5%-8% to 13% | Poor response to CIT, reduced overall survival | [16,17] |
FBXW7 mutations | Interferes with NOTCH1 signaling by degrading NOTCH1 intracellular domain by | 2% to 6% in newly diagnosed | Poor response to anti-CD20 antibody | [29,30] |
SF3B1 mutations | Impaired DNA damage response and increased NOTCH signaling | 3% to 17% | Poor response to CIT | [33-35] |
XPO1 mutations | Mislocalization of tumor suppressor proteins | Frequency similar to naïve and relapsed | Poor response to CIT | [37-39] |
RPS15 mutations | Increases TP53 degradation causing global changes towards a hyperproliferative cellular state | 5% to 20% | Poor response to CIT | [40-43] |
MGA mutations | Shifts balance towards MYC-dependent activation of cell proliferation and transformation | 3% to 16% | Poor response to CIT | [31,32] |
DNA damage response and cell cycle control
TP53
TP53 mutation and/or deletion (TP53 aberrancy) is the most important consideration when deciding about treatment using chemotherapy, since many studies, including prospective clinical trials, have shown poorer responses and shorter survival after chemotherapy treatment in patients with TP53 mutations or deletions of 17p[5-8]. TP53 encodes p53, a key mediator of the DNA damage response pathway that controls cell cycle arrest and apoptosis. Chemotherapy induces DNA damage, which, if not repaired by physiological DNA repairing enzymes, leads to a series of intracellular signaling related to TP53, causing cell apoptosis or preventing cell proliferation. TP53 aberrancies, by preventing apoptosis, result in the propagation of cells with an increasing number and type of chromosomal and genetic abnormalities. Targeted therapies attack specific genetic biomarkers independently of the p53 pathway and should thus be chosen in patients with known TP53 aberrancies. Deletions of chromosome 17p can be detected via fluorescence in situ hybridization (FISH), and an additional 5% of patients with CLL carry TP53 mutations without concomitant del(17p)[5,8]. The European Research Infrastructure Consortium (ERIC) guidelines suggest using Sanger sequencing (10%-20% sensitivity) or next-generation sequencing (NGS) to detect these TP53 mutations[9]. While the significance of low-burden TP53 mutations with < 10% VAF that are detected using NGS remains to be established[10], some studies have shown that chemoimmunotherapy leads to clonal expansion of small TP53 subclones, leading to a chemo-refractory phenotype with unfavorable prognosis at relapse[11]. In contrast, novel agents did not lead to the propagation of adverse subclones. In addition, CLL with TP53 abnormalities are associated with higher genomic complexity than CLL without, and this may have additional adverse prognostic implications for poor outcomes such as unmutated IGHV status, del(17p) or del(11q)[12].
Ataxia telangiectasia-mutated
The ataxia telangiectasia-mutated (ATM) gene maps to chromosome 11q22-q23 and ATM mutations are usually present at diagnosis in 20% of cases[13]. The ATM is a protein kinase that, following induction of DNA double-strand breaks, synchronizes DNA repair, activation of cell cycle checkpoints, and induction of apoptosis with elimination of cells with excessive DNA damage. In patients enrolled in the CLL4 trial treated with first-line fludarabine and cyclophosphamide, ATM mutation was associated with reduced overall and progression-free survival[14]. The residual ATM allele was mutated in 36% of cases of patients with 11q deletions, and biallelic ATM disruption led to complete loss of ATM function with the worst outcomes to CIT[15].
Protection of telomeres 1
The protection of telomeres 1 (POT1) encodes a protein member of the shelterin complex, which regulates telomere integrity. The shelterin complex compacts telomeric chromatin, which protects chromosome ends from instability, abnormal chromosome segregation, and inadequate recombination. Somatic mutations of the POT1 gene occur in 5%-8% of patients with CLL, affecting the ability of the protein to bind to telomeric DNA, leading to uncapping of telomere ends and chromosomal aberrations[16,17]. In the CLL11 trial, patients were randomized to receive chlorambucil, chlorambucil-rituximab, or chlorambucil-obinutuzumab. Patients with POT1 mutations receiving CIT had shorter overall survival (OS) independent of variables including Binet stage, elevated β2-microglobulin, unmutated IGHV status, and complex karyotype[16].
Nuclear factor-κB pathway
Baculoviral IAP repeat-containing protein 3
Baculoviral IAP repeat-containing protein 3 (BIRC3) is a negative regulator of non-canonical nuclear factor-κB (NF-κB) signaling, and inactivation or deletion of BIRC3 in CLL results in constitutive NF-κB pathway activation, which provides pro-survival signals to the leukemic clone through upregulation of several anti-apoptotic genes[18]. BIRC3 frameshift insertion/deletions or nonsense mutations are detected in around 4% of CLL patients at diagnosis, and since it is located on chromosome 11 in proximity to ATM, 11q deletions include the BIRC3 locus 83% of the time[14]. BIRC3 alterations are also associated with unmutated IGHV gene status and represent significant prognostic factors for unfavorable PFS after FCR and Obinutuzumab-chlorambucil[19-21]. Patients with BIRC3 mutations tended to have shorter overall survival compared with the wild-type group after treatment with CIT[22].
NOTCH signaling
NOTCH receptor 1
NOTCH receptor 1 (NOTCH1) codes for a transmembrane receptor that, upon binding to its ligand, is cleaved to generate transcriptionally active nuclear intracellular domain of NOTCH1, which transactivates genes involved in cell survival and proliferation, including myelocytomatosis oncogene (MYC) and components of the NF-κB pathway[23]. NOTCH1 mutations are present in 8%-12% of untreated patients, which can increase to 13%-21% in patients who are refractory to CIT[24]. NOTCH1 mutations are also enriched in patients with trisomy 12 and subset #1 and #8, which are associated with poor prognosis[25,26]. NOTCH1 mutated patients may not benefit from the addition of rituximab to FC due to lower cluster of differentiation 20 (CD20) expression leading to a lower extent of complement-dependent cytotoxicity induced by type I anti-CD20 monoclonal antibodies[24]. In CLL8, patients with mutated NOTCH1 treated with FC vs. FCR had a similar 5-year PFS rate of 25.8% vs. 26.7%[7]. Similarly, in a phase III trial evaluating chlorambucil alone against chlorambucil and ofatumumab, NOTCH1 mutations predicted refractoriness to ofatumumab[21]. However, obinutuzumab, a type 2 anti-CD20 monoclonal antibody with enhanced antibody-dependent cell-mediated cytotoxicity and increased direct cell-mediated cytotoxicity may retain efficacy in NOTCH1 mutated cases[27]. In the CLL11 trial, chlorambucil with obinutuzumab led to better progression-free and overall survival than chlorambucil with rituximab independent of NOTCH1 genetic lesions[28].
F-box and WD40 repeat domain containing-7 mutations
In CLL, F-box and WD40 repeat domain containing-7 (FBXW7) mutations occur at a frequency of 2% to 6% and commonly affect the WD40 domain of an E3 ubiquitin ligase that interacts with proteins that are subsequently subject to proteasomal degradation. Mutations of FBXW7 resulted in an increase of activated NOTCH1 intracellular domain (NICD) by preventing its degradation by proteasomes[29]. Thus, NICD accumulates in the nucleus thereby sustaining NOTCH1 target gene expression, leading to dysregulated NOTCH1 signaling without mutations. Among CLL patients who harbor NOTCH1 mutations, treatment with rituximab does not result in the expected increase in PFS compared with treatment with chemotherapy regimens that do not contain rituximab, and FBXW7 serves as another biomarker of CD20 monoclonal antibody resistance[30].
MYC deregulation
Max gene associated
Max gene associated (MGA) is a functional MYC suppressor that was found to be mutated in 3% of newly diagnosed CLL, 16% of fludarabine-refractory CLL, and 36% in Richter’s transformation[31]. MGA is a protein that forms a heterodimer with MAX and this heterodimer antagonizes MYC-dependent activation of transcription of target genes that drive cell proliferation, growth, and cellular transformation[32]. Genetic lesions including focal and recurrent gene deletions or truncating point mutations targeting MGA are enriched in high-risk CLL with chemoresistance and increase the tendency of transformation to an aggressive phenotype[32].
RNA and ribosomal processing pathway
SF3B1
SF3B1 encodes a component of the spliceosome, which is involved in the RNA splicing of precursor messenger RNA and the removal of introns in protein-encoding genes[33]. The SF3B1 gene is mutated in 3.6%-9.3% of patients with newly diagnosed CLL, which increases to 17% at relapse, and is enriched in patients with subset 2 disease[34]. Transcriptomic profiling revealed that multiple pathways are altered by SF3B1 mutations, including DNA damage response, cell cycle response, and NOTCH signaling[35]. SF3B1 mutations have been associated with fludarabine resistance and poor outcomes with shorter PFS in CLL8[33]. Moreover, co-expression of SF3B1-K700E with ATM deletion in murine B cells led to the onset of CLL, confirming the causative effects of this mutation in the onset of CLL[36].
Exportin 1
The exportin 1 (XPO1) gene is located on chromosome 2 and encodes a protein that mediates the translocation of numerous RNA and cellular regulatory proteins from the nucleus to the cytoplasm. The frequency of XPO1 mutations was similar in treatment-naïve and relapsed patients (~7.5%-10%); mutations lead to cytoplasmic mis-localization of tumor suppressor proteins (TP53), cell cycle inhibitors, and growth receptor proteins (e.g., STAT3)[37]. XPO1 mutations are associated with resistance to FCR and decreased PFS after chemoimmunotherapy, independent of IGHV mutation status or Binet stage[38]. Expression of Xpo1-E571K mutation in murine B cells resulted in the onset of CLL as early as 7 months of age[39]. Molecular characterization of these CLL cells revealed that mutant XPO1 altered the nucleo-cytoplasmic distribution of hundreds of proteins in a sequence-specific manner that promoted oncogenesis, rendering the sensitivity of these cells to inhibitors of nuclear export. These results provide a rationale for testing nuclear export inhibitor drugs in cases resistant to FCR.
Ribosomal protein S15
Ribosomal protein (RP) S15 is recurrently mutated in ~5% of untreated CLL, and mutations are associated with a reduced duration of response and resistance to fludarabine-based chemotherapy[40]. RPS15 mutations can affect up to 20% of CLL patients relapsing after FCR, and approximately a third of RPS15 mutated patients also carry a TP53 aberrancy[41]. Wild-type RPS15 binds to mouse double minute 2 homolog (MRM2) and inhibits its E3 ubiquitin ligase activity, abrogating p53 degradation, leading to upregulation of TP53 target genes, and cell apoptosis. Mutant RPS15 leads to increased TP53 degradation through ubiquitination and causes global changes of the proteome towards a hyperproliferative cellular state[42]. In the CLL8 trial, the mutation was associated with a shorter PFS, but with no significant reduction in OS[40]. In agreement with its critical role in driving aggressive type of CLL, co-expression of RPS15 mutation and TP53 deletion in murine B cells altered translation and MYC activation and led to the onset of B cell malignancy with a shortened latency compared to mice with RPS15 mutation alone[43].
MAPK-ERK pathway
KRAS and NRAS
The KRAS gene encodes a member of the GTPase superfamily and plays an important role in the regulation of cell proliferation. KRAS exon 2/3 mutations occur in ~6% of CLL and are associated with trisomy 12 CLL, promoting an increase in RAS/ERK downstream signaling and constitutive activation of the MAPK signaling pathway[44]. In the CLL11 trial, the presence of KRAS exon 2/3 mutation was associated with refractoriness to chemoimmunotherapy, especially to chlorambucil-rituximab[16]. The NRAS gene has intrinsic GTPase activity, is located at the 1p13.2 band, and encodes a membrane protein that alternates between the Golgi apparatus and the plasma membrane. The frequency of NRAS missense mutation in CLL patients is ~2.4%[44], mostly at subclonal levels. There is a mild association between the NRAS mutation and trisomy 12, and co-occurrence of NRAS with the KRAS mutation is associated with refractoriness to first-line treatment using chlorambucil-rituximab[16].
IGHV mutation status
The degree of somatic hypermutation of the immunoglobulin heavy-chain variable region (IGHV), which encodes part of the B-cell receptor, identifies two disease subtypes. Somatic hypermutation is a physiological process that generates immunoglobulin diversity during normal B cell maturation and remains stable throughout the course of the disease. IGHV is mutated ≥ 2% from a reference germline sequence in patients with mutated IGHV (M-CLL), while IGHV sequences are mutated less than 2% from germline sequence in patients with unmutated IGHV (U-CLL)[45]. U-CLL has increased B-cell receptor signaling capacity and poorer prognosis. Among patients receiving CIT, the IGHV mutation status affects the kinetics of relapse and thus PFS, and almost all U-CLL patients are projected to progress after chemoimmunotherapy[3,46]. Patients with U-CLL compared with M-CLL had worse outcomes after FCR in CLL8 (5-year PFS 33.1% vs. 66.6%), after bendamustine-rituximab in CLL10 (median PFS 33.9 vs. 68.9 months), and after chlorambucil-based regimens in CLL11[47].
Transcriptome
Gene expression clusters
While the current classification of CLL still relies largely on genomic alterations, novel insights from nongenomic RNA sequencing data have led to consensus clustering of gene expression subgroups with unique clinical and biological significance. In a recent study by Knisbacher et al., where whole-exome sequencing and whole-genome sequencing data were analyzed from 1,074 CLL cases, the authors defined eight gene expression clusters (EC) with characteristic transcriptomic profiles and differing prognoses[48].
MicroRNA
MicroRNAs are short non-coding RNAs that modulate post-transcriptional gene expression and are involved in the regulation of many physiological and pathological processes. Several studies identified microRNA expression profiles in CLL cells that were associated with resistance to fludarabine and rituximab. Both lower expression of miR-34a and high miR-155 expression have been associated with poor response to fludarabine[50-52]. miR-34a is a microRNA component of the p53 pathway and directly affects CDK4, CDK6, CCND1, CCNE2, and MET proto-oncogene[53]. A high expression level of miRNA-155 can attenuate histone deacetylase 4 and BCL6 transcription repressor expression, leading to the activation of oncogenes associated with increased cell division and apoptosis inhibition[54].
RNA splicing
Dysregulated RNA splicing is a prominent molecular trait found across various tumor types. Splicing alterations in cancer stem from recurrent mutations and changes in the expression of regulatory factors responsible for splicing control. RNA splicing dysregulation can drive tumorigenesis through a multitude of mechanisms, including promoting cell proliferation, inhibiting apoptosis, enhancing migration and metastasis, fostering resistance to chemotherapy, and enabling evasion of the immune system’s surveillance. In CLL, mutations in splicing factors SF3B1 and U1snRNA have been reported previously and are linked to poor prognosis. CLL cells with these mutations all have transcriptome-wide splicing changes[55,56]. In addition, recent studies also revealed that RNA splicing dysregulation in CLL cannot be fully explained by genetic alterations of the splicing factor SF3B1 alone but is contributed by upregulation of splicing factors at the protein level compared to normal B cells. This could be caused by post-transcriptional regulation in CLL cells, such as RNA epigenetic modification[57]. More importantly, splice variants can be used to stratify CLL patients into indolent and aggressive subgroups[58]. It remains elusive how RNA splicing dysregulation is related to FCR resistance and whether RNA splicing inhibitors currently in clinical trials may override the resistance.
Epigenome
DNA methylation change subgroup
Epigenetics comprises modifications of the DNA molecule that affect gene expression through transcription, without changes to the nucleic acid sequence. Epigenetic mechanisms include DNA methylation, histone modifications, chromatin accessibility, and gene regulation through non-coding RNAs. DNA methylation leads to the addition of a methyl group to 5’carbon of cytosine in cytosine guanine dinucleotides (CpG), and is the most extensively studied epigenetic change studied in CLL. CLL has been clustered into three epigenetic subgroups based on DNA methylation profiles, as follows: naïve B-cell like CLL (n-CLL), memory B-cell like CLL (M-CLL), and intermediate group (i-CLL) from antigen-exposed B-cell that has not passed the germinal center[59-61]. The majority (97%) of n-CLL have unmutated IGHV genes, while most M-CLL have mutated IGHV. The i-CLL group has a borderline IGHV mutational load and showed higher (38%) use of IGLV3-21R110 mutation which promotes autonomous B-cell receptor signaling. While these patients have borderline mutated IGHV genes, their phenotype resembles unmutated CLL, with aggressive disease characteristics and adverse clinical outcomes with CIT[62]. In addition, a higher cumulative mitoses (epiCMIT) score within individual epitypes, which reflects a more extensive CLL proliferation history, was associated with a worse prognosis[63]. Another epigenetic biomarker identified was DNA hypermethylation at HOXA4. Both HOXA4 hypermethylation during CLL progression and relapse and loss of HOXA4 expression were associated with resistance to chemotherapeutic agents including fludarabine[64].
RNA epigenetic modification
Increasing evidence emphasizes the crucial functions of N6-methyladenosine (m6A) modification in mRNA during cancer initiation, progression, and drug resistance. Nevertheless, the roles of m6A in CLL remain largely unexplored. Recent findings from our group suggest that CLL cells exhibit elevated expression of METTL3 protein, an RNA methyltransferase responsible for depositing m6A on mRNA. Correspondingly, there is also an upregulation of m6A on mRNA in CLL cells. Notably, a higher abundance of METTL3 protein correlates with the need for early treatment in CLL patients. Further investigations into the molecular mechanism of METTL3 in CLL have revealed its involvement in regulating the RNA splicing network, and its association with CLL aggressiveness. Subsequent analysis of FCR resistance will illustrate whether m6A modification and RNA splicing dysregulation may play a role in this aspect[57].
Clonal and subclonal evolution
Genetic lesions in CLL may change over time, leading to clonal evolution and the emergence of more aggressive clones or subclones. Clonal evolution is often accelerated in cells treated with chemotherapy that induces DNA damage, leading to the accumulation of genetic aberrations and increased genetic complexity, promoting progression and chemorefractoriness. Recent genomic studies have demonstrated significant intratumoral heterogeneity within CLL that is characterized by the presence of genetically diverse clones and subclones that interact and compete. Clones and subclones carrying genetic lesions that resulted from genotoxic chemotherapy, along with TP53 aberrancies that prevent DNA repair, are resistant to and selected by CIT. As a result, clonal evolution occurs more frequently in patients with CLL receiving CIT, while clonal architecture remains stable in untreated CLL or in CLL treated by targeted therapy[65,66].
DISCUSSION AND CONCLUSIONS
FCR remains a viable option for long-term durable remission in patients with IGHV mutated disease without del(11q) or del(17p) who desire a short therapy course, after proper counseling of the not insignificant risk of therapy-related myeloid neoplasm (6.3%, 84% of which are fatal events). Recently, a
DECLARATIONS
Authors’ contributions
Performed the literature review and wrote the initial draft: Ong SY
Reviewed and revised the manuscript: Wang L
Availability of data and materials
Not applicable.
Financial support and sponsorship
None.
Conflicts of interest
Both authors declared that there are no conflicts of interest.
Ethical approval and consent to participate
Not applicable.
Consent for publication
Not applicable.
Copyright
© The Author(s) 2024.
REFERENCES
1. Chen Q, Jain N, Ayer T, et al. Economic burden of chronic lymphocytic leukemia in the era of oral targeted therapies in the United States. J Clin Oncol 2017;35:166-74.
2. Thompson PA, Tam CS, O’Brien SM, et al. Fludarabine, cyclophosphamide, and rituximab treatment achieves long-term disease-free survival in IGHV-mutated chronic lymphocytic leukemia. Blood 2016;127:303-9.
3. Fischer K, Bahlo J, Fink AM, et al. Long-term remissions after FCR chemoimmunotherapy in previously untreated patients with CLL: updated results of the CLL8 trial. Blood 2016;127:208-15.
4. Eichhorst B, Niemann CU, Kater AP, et al. GCLLSG; the HOVON and Nordic CLL Study Groups; the SAKK; the Israeli CLL Association; and Cancer Trials Ireland. First-line venetoclax combinations in chronic lymphocytic leukemia. N Engl J Med 2023;388:1739-54.
5. Zenz T, Eichhorst B, Busch R, et al. TP53 mutation and survival in chronic lymphocytic leukemia. J Clin Oncol 2010;28:4473-9.
6. Fischer K, Cramer P, Busch R, et al. Bendamustine in combination with rituximab for previously untreated patients with chronic lymphocytic leukemia: a multicenter phase II trial of the German Chronic Lymphocytic Leukemia Study Group. J Clin Oncol 2012;30:3209-16.
7. Stilgenbauer S, Schnaiter A, Paschka P, et al. Gene mutations and treatment outcome in chronic lymphocytic leukemia: results from the CLL8 trial. Blood 2014;123:3247-54.
8. Rossi D, Cerri M, Deambrogi C, et al. The prognostic value of TP53 mutations in chronic lymphocytic leukemia is independent of Del17p13: implications for overall survival and chemorefractoriness. Clin Cancer Res 2009;15:995-1004.
9. Malcikova J, Tausch E, Rossi D, et al. European Research Initiative on Chronic Lymphocytic Leukemia (ERIC) -
11. Malcikova J, Pavlova S, Kunt Vonkova B, et al. Low-burden TP53 mutations in CLL: clinical impact and clonal evolution within the context of different treatment options. Blood 2021;138:2670-85.
12. Rigolin GM, Cavallari M, Quaglia FM, et al. In CLL, comorbidities and the complex karyotype are associated with an inferior outcome independently of CLL-IPI. Blood 2017;129:3495-8.
13. Raponi S, Del Giudice I, Marinelli M, et al. Genetic landscape of ultra-stable chronic lymphocytic leukemia patients. Ann Oncol 2018;29:966-72.
14. Rose-Zerilli MJ, Forster J, Parker H, et al. ATM mutation rather than BIRC3 deletion and/or mutation predicts reduced survival in 11q-deleted chronic lymphocytic leukemia: data from the UK LRF CLL4 trial. Haematologica 2014;99:736-42.
15. Austen B, Skowronska A, Baker C, et al. Mutation status of the residual ATM allele is an important determinant of the cellular response to chemotherapy and survival in patients with chronic lymphocytic leukemia containing an 11q deletion. J Clin Oncol 2007;25:5448-57.
16. Herling CD, Klaumünzer M, Rocha CK, et al. Complex karyotypes and KRAS and POT1 mutations impact outcome in CLL after chlorambucil-based chemotherapy or chemoimmunotherapy. Blood 2016;128:395-404.
17. Ramsay AJ, Quesada V, Foronda M, et al. POT1 mutations cause telomere dysfunction in chronic lymphocytic leukemia. Nat Genet 2013;45:526-30.
18. Asslaber D, Wacht N, Leisch M, et al. BIRC3 expression predicts CLL progression and defines treatment sensitivity via enhanced
19. Diop F, Moia R, Favini C, et al. Biological and clinical implications of BIRC3 mutations in chronic lymphocytic leukemia. Haematologica 2020;105:448-56.
20. Kater AP, Jiang Y, Chyla B, Seymour JF. Response in patients with BIRC3-mutated relapsed/refractory chronic lymphocytic leukemia treated with fixed-duration venetoclax and rituximab. Haematologica 2020;105:e382-3.
21. Tausch E, Beck P, Schlenk RF, et al. Prognostic and predictive role of gene mutations in chronic lymphocytic leukemia: results from the pivotal phase III study COMPLEMENT1. Haematologica 2020;105:2440-7.
22. Nadeu F, Delgado J, Royo C, et al. Clinical impact of clonal and subclonal TP53, SF3B1, BIRC3, NOTCH1, and ATM mutations in chronic lymphocytic leukemia. Blood 2016;127:2122-30.
23. Fabbri G, Holmes AB, Viganotti M, et al. Common nonmutational NOTCH1 activation in chronic lymphocytic leukemia. Proc Natl Acad Sci U S A 2017;114:E2911-9.
24. Rossi D, Rasi S, Fabbri G, et al. Mutations of NOTCH1 are an independent predictor of survival in chronic lymphocytic leukemia. Blood 2012;119:521-9.
25. Balatti V, Bottoni A, Palamarchuk A, et al. NOTCH1 mutations in CLL associated with trisomy 12. Blood 2012;119:329-31.
26. Sutton LA, Young E, Baliakas P, et al. ERIC; the European Research Initiative on CLL. Different spectra of recurrent gene mutations in subsets of chronic lymphocytic leukemia harboring stereotyped B-cell receptors. Haematologica 2016;101:959-67.
27. Edelmann J, Dokal AD, Vilventhraraja E, et al. Rituximab and obinutuzumab differentially hijack the B cell receptor and NOTCH1 signaling pathways. iScience 2021;24:102089.
28. Estenfelder S, Tausch E, Robrecht S, et al. Gene mutations and treatment outcome in the context of chlorambucil (Clb) without or with the addition of Rituximab (R) or Obinutuzumab (GA-101, G) - results of an extensive analysis of the phase III study CLL11 of the German CLL Study Group. Blood 2016;128:3227.
29. Close V, Close W, Kugler SJ, et al. FBXW7 mutations reduce binding of NOTCH1, leading to cleaved NOTCH1 accumulation and target gene activation in CLL. Blood 2019;133:830-9.
31. De Paoli L, Cerri M, Monti S, et al. MGA, a suppressor of MYC, is recurrently inactivated in high risk chronic lymphocytic leukemia. Leuk Lymphoma 2013;54:1087-90.
32. Hurlin PJ, Steingrìmsson E, Copeland NG, Jenkins NA, Eisenman RN. Mga, a dual-specificity transcription factor that interacts with Max and contains a T-domain DNA-binding motif. EMBO J 2000;19:3841-2.
33. Rossi D, Bruscaggin A, Spina V, et al. Mutations of the SF3B1 splicing factor in chronic lymphocytic leukemia: association with progression and fludarabine-refractoriness. Blood 2011;118:6904-8.
35. Wang L, Brooks AN, Fan J, et al. Transcriptomic characterization of SF3B1 mutation reveals its pleiotropic effects in chronic lymphocytic leukemia. Cancer Cell 2016;30:750-63.
36. Yin S, Gambe RG, Sun J, et al. A murine model of chronic lymphocytic leukemia based on B cell-restricted expression of Sf3b1 mutation and atm deletion. Cancer Cell 2019;35:283-96.e5.
37. Walker JS, Hing ZA, Harrington B, et al. Recurrent XPO1 mutations alter pathogenesis of chronic lymphocytic leukemia. J Hematol Oncol 2021;14:17.
38. Cosson A, Chapiro E, Bougacha N, et al. Gain in the short arm of chromosome 2 (2p+) induces gene overexpression and drug resistance in chronic lymphocytic leukemia: analysis of the central role of XPO1. Leukemia 2017;31:1625-9.
39. Taylor J, Sendino M, Gorelick AN, et al. Altered nuclear export signal recognition as a driver of oncogenesis. Cancer Discov 2019;9:1452-67.
40. Landau DA, Tausch E, Taylor-Weiner AN, et al. Mutations driving CLL and their evolution in progression and relapse. Nature 2015;526:525-30.
41. Ljungström V, Cortese D, Young E, et al. Whole-exome sequencing in relapsing chronic lymphocytic leukemia: clinical impact of recurrent RPS15 mutations. Blood 2016;127:1007-16.
42. Bretones G, Álvarez MG, Arango JR, et al. Altered patterns of global protein synthesis and translational fidelity in RPS15-mutated chronic lymphocytic leukemia. Blood 2018;132:2375-88.
43. Gutierrez C, Ouspenskaia T, Fu D, et al. RPS15 and TP53 co-mutation drives B cell malignancy through altered translation and MYC activation in a murine model. Blood 2020;136:28-9.
44. Vendramini E, Bomben R, Pozzo F, et al. KRAS, NRAS, and BRAF mutations are highly enriched in trisomy 12 chronic lymphocytic leukemia and are associated with shorter treatment-free survival. Leukemia 2019;33:2111-5.
45. Ghia P, Stamatopoulos K, Belessi C, et al. European Research Initiative on CLL. ERIC recommendations on IGHV gene mutational status analysis in chronic lymphocytic leukemia. Leukemia 2007;21:1-3.
46. Eichhorst B, Fink AM, Bahlo J, et al. international group of investigators; German CLL Study Group (GCLLSG). First-line chemoimmunotherapy with bendamustine and rituximab versus fludarabine, cyclophosphamide, and rituximab in patients with advanced chronic lymphocytic leukaemia (CLL10): an international, open-label, randomised, phase 3, non-inferiority trial. Lancet Oncol 2016;17:928-42.
47. Langerak AW, Ritgen M, Goede V, et al. Prognostic value of MRD in CLL patients with comorbidities receiving chlorambucil plus obinutuzumab or rituximab. Blood 2019;133:494-7.
48. Knisbacher BA, Lin Z, Hahn CK, et al. Molecular map of chronic lymphocytic leukemia and its impact on outcome. Nat Genet 2022;54:1664-74.
49. Herling CD, Coombes KR, Benner A, et al. Time-to-progression after front-line fludarabine, cyclophosphamide, and rituximab chemoimmunotherapy for chronic lymphocytic leukaemia: a retrospective, multicohort study. Lancet Oncol 2019;20:1576-86.
50. Ferracin M, Zagatti B, Rizzotto L, et al. MicroRNAs involvement in fludarabine refractory chronic lymphocytic leukemia. Mol Cancer 2010;9:123.
51. Ferrajoli A, Shanafelt TD, Ivan C, et al. Prognostic value of miR-155 in individuals with monoclonal B-cell lymphocytosis and patients with B chronic lymphocytic leukemia. Blood 2013;122:1891-9.
52. Zenz T, Mohr J, Eldering E, et al. miR-34a as part of the resistance network in chronic lymphocytic leukemia. Blood 2009;113:3801-8.
53. He L, He X, Lowe SW, Hannon GJ. microRNAs join the p53 network - another piece in the tumour-suppression puzzle. Nat Rev Cancer 2007;7:819-22.
54. Calin GA, Liu CG, Sevignani C, et al. MicroRNA profiling reveals distinct signatures in B cell chronic lymphocytic leukemias. Proc Natl Acad Sci U S A 2004;101:11755-60.
55. Wang L, Lawrence MS, Wan Y, et al. SF3B1 and other novel cancer genes in chronic lymphocytic leukemia. N Engl J Med 2011;365:2497-506.
56. Shuai S, Suzuki H, Diaz-Navarro A, et al. The U1 spliceosomal RNA is recurrently mutated in multiple cancers. Nature 2019;574:712-6.
57. Wu Y, Jin M, Fernandez M, et al. METTL3-mediated m6A modification controls splicing factor abundance and contributes to aggressive CLL. Blood Cancer Discov 2023;4:228-45.
58. Herbst SA, Vesterlund M, Helmboldt AJ, et al. Proteogenomics refines the molecular classification of chronic lymphocytic leukemia. Nat Commun 2022;13:6226.
59. Kulis M, Heath S, Bibikova M, et al. Epigenomic analysis detects widespread gene-body DNA hypomethylation in chronic lymphocytic leukemia. Nat Genet 2012;44:1236-42.
60. Oakes CC, Seifert M, Assenov Y, et al. DNA methylation dynamics during B cell maturation underlie a continuum of disease phenotypes in chronic lymphocytic leukemia. Nat Genet 2016;48:253-64.
61. Queirós AC, Villamor N, Clot G, et al. A B-cell epigenetic signature defines three biologic subgroups of chronic lymphocytic leukemia with clinical impact. Leukemia 2015;29:598-605.
62. Nadeu F, Royo R, Clot G, et al. IGLV3-21R110 identifies an aggressive biological subtype of chronic lymphocytic leukemia with intermediate epigenetics. Blood 2021;137:2935-46.
63. Duran-Ferrer M, Clot G, Nadeu F, et al. The proliferative history shapes the DNA methylome of B-cell tumors and predicts clinical outcome. Nat Cancer 2020;1:1066-81.
64. Barrow TM, Nakjang S, Lafta F, et al. Epigenome-wide analysis reveals functional modulators of drug sensitivity and post-treatment survival in chronic lymphocytic leukaemia. Br J Cancer 2021;124:474-83.
65. Gruber M, Bozic I, Leshchiner I, et al. Growth dynamics in naturally progressing chronic lymphocytic leukaemia. Nature 2019;570:474-9.
66. Landau DA, Sun C, Rosebrock D, et al. The evolutionary landscape of chronic lymphocytic leukemia treated with ibrutinib targeted therapy. Nat Commun 2017;8:2185.
Cite This Article
Export citation file: BibTeX | RIS
OAE Style
Ong SY, Wang L. Leveraging genomics, transcriptomics and epigenomics to understand chemoimmunotherapy resistance in chronic lymphocytic leukemia. Cancer Drug Resist 2024;7:7. http://dx.doi.org/10.20517/cdr.2023.98
AMA Style
Ong SY, Wang L. Leveraging genomics, transcriptomics and epigenomics to understand chemoimmunotherapy resistance in chronic lymphocytic leukemia. Cancer Drug Resistance. 2024; 7: 7. http://dx.doi.org/10.20517/cdr.2023.98
Chicago/Turabian Style
Ong, Shin Yeu, Lili Wang. 2024. "Leveraging genomics, transcriptomics and epigenomics to understand chemoimmunotherapy resistance in chronic lymphocytic leukemia" Cancer Drug Resistance. 7: 7. http://dx.doi.org/10.20517/cdr.2023.98
ACS Style
Ong, SY.; Wang L. Leveraging genomics, transcriptomics and epigenomics to understand chemoimmunotherapy resistance in chronic lymphocytic leukemia. Cancer Drug Resist. 2024, 7, 7. http://dx.doi.org/10.20517/cdr.2023.98
About This Article
Special Issue
Copyright
Data & Comments
Data
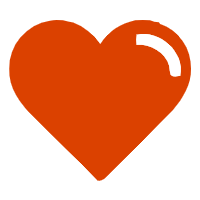

Comments
Comments must be written in English. Spam, offensive content, impersonation, and private information will not be permitted. If any comment is reported and identified as inappropriate content by OAE staff, the comment will be removed without notice. If you have any queries or need any help, please contact us at support@oaepublish.com.